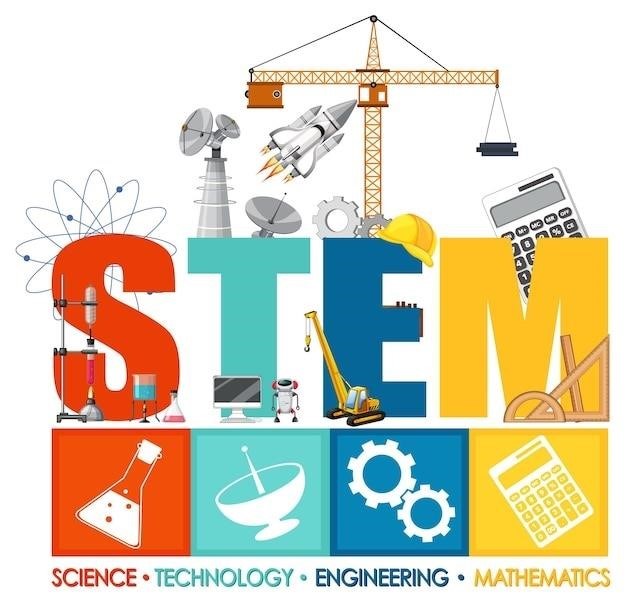
Fundamentals of Engineering Thermodynamics⁚ A Comprehensive Overview
This article provides a comprehensive overview of the fundamentals of engineering thermodynamics, covering key concepts, definitions, and applications. It explores the fundamental laws of thermodynamics, including the first, second, and third laws, and their relevance to engineering systems; The article also delves into thermodynamic systems, properties, work, heat, and thermodynamic cycles. It concludes with a discussion of the applications of thermodynamics in various engineering fields, highlighting its significance in solving real-world problems.
Introduction to Engineering Thermodynamics
Engineering thermodynamics is a fundamental branch of engineering that deals with the study of energy, its transformation, and its applications in various engineering systems. It provides a framework for understanding and predicting the behavior of energy in physical processes, enabling engineers to design and optimize systems for efficient energy utilization. Thermodynamics is essential for understanding and analyzing a wide range of engineering applications, including power generation, refrigeration, heat transfer, combustion, and chemical reactions. This field is built upon a set of fundamental laws that govern energy interactions and transformations. These laws, often referred to as the first, second, and third laws of thermodynamics, form the foundation of the subject and are universally applicable.
The study of engineering thermodynamics involves understanding the concepts of heat, work, temperature, entropy, and energy. These concepts are used to analyze and predict the behavior of systems in various states and processes. Thermodynamics also explores the relationship between energy and its various forms, including mechanical, thermal, chemical, and electrical energy, and how these forms can be converted from one to another. The principles of thermodynamics are essential for understanding the efficiency and limitations of energy conversion processes, leading to the development of more efficient and sustainable energy technologies.
Basic Concepts and Definitions
The study of engineering thermodynamics is built upon a foundation of fundamental concepts and definitions that are crucial for understanding the behavior of energy in various systems. These basic concepts provide a framework for analyzing and predicting energy transformations and interactions. Some key concepts include⁚
- System⁚ A system is a defined region of space that is under consideration for thermodynamic analysis. It can be a closed system, where no mass transfer occurs across its boundaries, or an open system, where mass and energy can cross the boundaries.
- Surroundings⁚ The surroundings refer to everything outside the system that can interact with it.
- Boundary⁚ The boundary separates the system from its surroundings, defining the limits of the system.
- Property⁚ A property is a characteristic of a system that can be measured or calculated, such as temperature, pressure, volume, and energy.
- State⁚ The state of a system is defined by the values of its properties at a particular instant in time.
- Process⁚ A process is a change in the state of a system, involving changes in its properties.
- Equilibrium⁚ Equilibrium refers to a state where the properties of a system remain constant over time. There are different types of equilibrium, including thermal, mechanical, and chemical equilibrium.
- Heat⁚ Heat is a form of energy transfer that occurs due to a temperature difference between a system and its surroundings.
- Work⁚ Work is a form of energy transfer that occurs when a force acts over a distance.
These basic concepts provide the foundation for understanding and applying the principles of thermodynamics in various engineering applications.
Thermodynamic Systems and Properties
Thermodynamic systems are the central focus of engineering thermodynamics. They are defined regions of space that are under investigation for their energy interactions and transformations. Understanding the characteristics of these systems and their properties is essential for analyzing and predicting their behavior.
Thermodynamic systems can be categorized into three main types⁚
- Closed System⁚ A closed system is one that exchanges energy with its surroundings but does not exchange mass. This means that the system’s mass remains constant throughout a process. Examples include a sealed container filled with gas or a piston-cylinder arrangement.
- Open System⁚ An open system exchanges both energy and mass with its surroundings. This means that the system’s mass can change during a process. Examples include a turbine, a compressor, or a combustion chamber.
- Isolated System⁚ An isolated system does not exchange energy or mass with its surroundings. It is a theoretical concept, as true isolation is difficult to achieve in practice.
Each thermodynamic system possesses specific properties that describe its state. These properties can be extensive, meaning they depend on the system’s size, or intensive, meaning they are independent of the system’s size. Common thermodynamic properties include⁚
- Temperature (T)⁚ A measure of the average kinetic energy of the molecules within a system.
- Pressure (P)⁚ The force exerted per unit area by a system on its surroundings.
- Volume (V)⁚ The space occupied by a system.
- Internal Energy (U)⁚ The total energy stored within a system due to the motion and interaction of its molecules.
- Enthalpy (H)⁚ A thermodynamic property that combines internal energy and the product of pressure and volume.
- Entropy (S)⁚ A measure of the randomness or disorder within a system.
Understanding these properties and their relationships is crucial for analyzing and predicting the behavior of thermodynamic systems in various engineering applications.
Work and Heat
Work and heat are crucial concepts in thermodynamics, representing the modes of energy transfer between a system and its surroundings. They are distinct from internal energy, which is the energy contained within the system itself.
Work (W) is defined as the energy transferred to or from a system due to a force acting over a distance. It is a form of energy that is transferred as a result of the system’s interaction with its surroundings. Work can be positive (done by the system) or negative (done on the system).
Examples of work include⁚
- Expansion or Compression Work⁚ When a system expands or contracts against an external pressure, work is done.
- Shaft Work⁚ Work done by a rotating shaft, such as in a turbine or pump.
- Electrical Work⁚ Work done by an electric current flowing through a system.
Heat (Q) is defined as the energy transferred to or from a system due to a temperature difference between the system and its surroundings. It is a form of energy transfer that occurs spontaneously from a region of higher temperature to a region of lower temperature.
Heat can be positive (heat added to the system) or negative (heat removed from the system). Examples of heat transfer include⁚
- Conduction⁚ Heat transfer through direct contact between objects at different temperatures.
- Convection⁚ Heat transfer through the movement of fluids (liquids or gases).
- Radiation⁚ Heat transfer through electromagnetic waves.
In summary, work and heat are distinct forms of energy transfer that play a crucial role in thermodynamic processes. Understanding their mechanisms and their impact on the energy balance of systems is fundamental for solving engineering problems related to energy conversion and utilization.
The First Law of Thermodynamics
The First Law of Thermodynamics is a fundamental principle that governs the conservation of energy. It states that energy cannot be created or destroyed, but can only be transformed from one form to another. In other words, the total energy of an isolated system remains constant.
The First Law can be expressed mathematically as follows⁚
ΔU = Q ⏤ W
Where⁚
- ΔU is the change in internal energy of the system
- Q is the heat added to the system
- W is the work done by the system
This equation implies that the change in internal energy of a system is equal to the heat added to the system minus the work done by the system. If heat is added to the system (Q > 0), the internal energy increases (ΔU > 0). Conversely, if work is done by the system (W > 0), the internal energy decreases (ΔU < 0).
The First Law has several important implications for engineering systems⁚
- Energy Conservation⁚ It emphasizes the importance of energy conservation in engineering design and operation.
- Energy Conversion⁚ It provides a framework for understanding and analyzing energy conversion processes, such as in power plants and engines.
- Thermodynamic Cycles⁚ It forms the basis for analyzing thermodynamic cycles, which are used in various engineering applications.
In conclusion, the First Law of Thermodynamics is a fundamental principle that governs the conservation of energy. It has wide-ranging implications for engineering systems, providing a framework for understanding energy conversion, energy conservation, and the analysis of thermodynamic cycles.
The Second Law of Thermodynamics
The Second Law of Thermodynamics is another fundamental principle that governs the direction of energy transfer and the behavior of thermodynamic systems. It states that heat cannot spontaneously flow from a colder body to a hotter body. This implies that natural processes tend to move towards a state of greater disorder or entropy.
The Second Law can be expressed in several different forms, including⁚
- Kelvin-Planck Statement⁚ It is impossible to construct a device that operates in a cycle and produces no effect other than the transfer of heat from a single reservoir and the performance of an equivalent amount of work.
- Clausius Statement⁚ It is impossible to construct a device that operates in a cycle and whose sole effect is the transfer of heat from a colder body to a hotter body.
- Entropy Statement⁚ The entropy of an isolated system always increases over time, or remains constant in reversible processes.
The Second Law has significant implications for engineering systems⁚
- Efficiency Limitations⁚ It sets limits on the efficiency of energy conversion processes, such as in power plants and engines. For example, the Carnot cycle, a theoretical thermodynamic cycle, establishes the maximum possible efficiency for a heat engine operating between two temperatures.
- Irreversibility⁚ It explains the irreversibility of natural processes, meaning that processes cannot be reversed without leaving some change in the surroundings. This concept is essential for understanding the performance of real-world systems.
- Entropy and Design⁚ Entropy considerations are crucial in engineering design, particularly in optimizing system performance and minimizing energy losses.
In conclusion, the Second Law of Thermodynamics is a fundamental principle that governs the direction of energy transfer and the behavior of thermodynamic systems. It has significant implications for engineering systems, setting limits on efficiency, explaining irreversibility, and guiding design considerations.
Entropy and the Third Law of Thermodynamics
Entropy, a fundamental concept in thermodynamics, represents a measure of disorder or randomness within a system. It is a state function, meaning its value depends solely on the current state of the system and not on the path taken to reach that state. Entropy increases as the system becomes more disordered, indicating a greater number of possible microstates or arrangements of its constituent particles.
The Third Law of Thermodynamics builds upon the concept of entropy, stating that the entropy of a perfect crystal at absolute zero (0 Kelvin) is zero. This implies that at absolute zero, the system exists in a state of perfect order and minimal energy. The Third Law has several important implications⁚
- Entropy as a Limit⁚ It defines a lower limit for entropy, meaning that entropy can never be negative. As a system approaches absolute zero, its entropy approaches zero, but it can never reach a negative value. This concept is essential for understanding the behavior of matter at extremely low temperatures.
- Unattainable Absolute Zero⁚ The Third Law implies that reaching absolute zero is impossible, as it would require a perfectly ordered state, which is practically unattainable. This concept is critical in the field of cryogenics, which involves the study and production of extremely low temperatures.
- Thermodynamic Equilibrium⁚ The Third Law is closely related to the concept of thermodynamic equilibrium, which occurs when a system reaches a state of maximum entropy and minimal energy. This state represents a state of balance and stability within the system.
In summary, entropy and the Third Law of Thermodynamics are fundamental concepts that provide insights into the behavior of thermodynamic systems. They establish a lower limit for entropy, explain the unattainability of absolute zero, and connect to the concept of thermodynamic equilibrium. These principles are crucial for understanding and analyzing various engineering systems, particularly those operating at low temperatures.
Thermodynamic Cycles
Thermodynamic cycles are a series of interconnected processes that return a system to its initial state, allowing for continuous operation and the transfer of energy. These cycles are fundamental to various engineering applications, such as power generation, refrigeration, and heat pumps. Each cycle consists of specific processes, typically involving heat transfer, work, and changes in internal energy.
Common thermodynamic cycles include⁚
- Carnot Cycle⁚ This theoretical cycle represents the most efficient possible cycle operating between two temperature reservoirs. It consists of four reversible processes⁚ isothermal expansion, adiabatic expansion, isothermal compression, and adiabatic compression. The Carnot cycle serves as a benchmark for evaluating the efficiency of real-world cycles.
- Rankine Cycle⁚ This cycle is widely used in power plants, particularly those using steam turbines. It involves four processes⁚ water is heated to generate steam, the steam expands through a turbine to produce work, the steam is condensed, and the condensed water is pumped back to the boiler. The Rankine cycle is a practical cycle that provides a balance between efficiency and practicality.
- Otto Cycle⁚ This cycle is commonly used in spark-ignition internal combustion engines. It involves four processes⁚ intake, compression, combustion, and exhaust. The Otto cycle describes the operation of gasoline engines and other similar engines.
- Diesel Cycle⁚ This cycle is used in diesel engines. It resembles the Otto cycle but differs in the combustion process, which occurs at a constant pressure rather than constant volume. The Diesel cycle is known for its higher compression ratios and ability to use lower-grade fuels.
Understanding thermodynamic cycles is crucial for designing and analyzing various engineering systems. These cycles provide a framework for analyzing the efficiency and performance of energy conversion processes and optimizing their operation for specific applications.